SPECTROMETER BASICS
Tutorial Explaining How Spectrometer's Work & How to Select a Spectrometer

Introduction:
Our understanding of the universe comes from an expanded understanding of light as a wave across a much wider spectrum than what we can see. This understanding has led to a host of modern technologies utilizing light’s wave properties to transmit information (audio and visual communications), and to measure spectral components of physical phenomena such as mass of particles, and the chemical make-up of matter both terrestrial and extraterrestrial. More »
QUICK NAVIGATION:
- SHOP SPECTROMETERS AND ACCESSORIES
- WHAT IS SPECTROSCOPY?
- HOW DOES A SPECTROMETER WORK?
- MAIN COMPONENTS OF A SPECTROMETER
- TYPES OF OPTICAL PATHS IN SPECTROMETERS
- WHAT IS FLUORESCENCE SPECTROSCOPY?
- WHAT IS ABSORPTION SPECTROSCOPY?
- WHAT IS RAMAN SPECTROSCOPY?
SHOP POPULAR SPECTROMETERS AND ACCESSORIES
What is Spectroscopy?
Spectroscopy is a scientific measurement technique that investigates and quantifies the interaction of a light source with matter. Several types of light-matter interactions at the molecular level can be measured including emission, absorption, fluorescence and scattering. These interactions are qualitatively and quantitatively analyzed with light measuring instruments; the qualitative analysis is used to establish the identity of a sample while a quantitative analysis is performed to estimate the concentration of a substance. Every substance and element interacts differently and uniquely with light frequencies, the resulting patterns act like their own signatures or fingerprints.
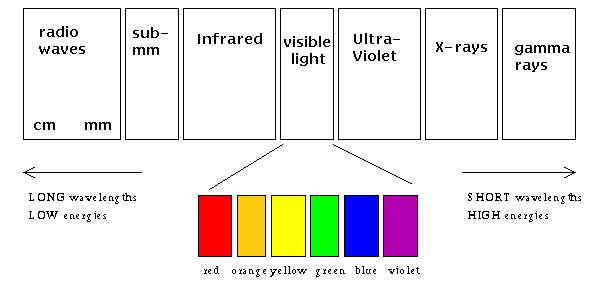
Physicists classify light waves by their respective energy (wavelength), hence, the electromagnetic spectrum. Short wavelength light is high energy, while long wavelength light is low energy. How we “see” this light energy is through an instrument called a spectrometer. Spectrometers can and are used in all of the physical sciences; physics, chemistry, biology, astronomy, geology, metrology among others over thousands of applications in communications, energy, pharmaceutical, health care, agricultural, manufacturing, safety and security just to name a few. Spectroscopy is a quiet revolution that has substantially changed and affected the way we interact with and better understand our modern world.
This article is a discussion of a relatively recent subset of compact to miniature spectrometers that have enabled science to be conducted outside the lab for on-site analysis. These incorporate a wavelength dispersive element known as a diffraction grating covering light wavelengths from the UV to IR spectrum.
What is the function of the Optical Spectrometer?
The spectrometer is now a common scientific instrument used to determine characteristic information about an object and/or element (sample) through analysis of its interactions with light or of the spectral components of light sources themselves. They leverage light’s wavelike properties to produce a spectrum and then measure the characteristics of the spectrum, i.e., wavelength, frequency and intensity. That information is processed by a computer for correlation and display. They can function over any range of the electromagnetic spectrum, but through design and selection of appropriate optical components, they typically operate in an application dependent region of the spectrum.
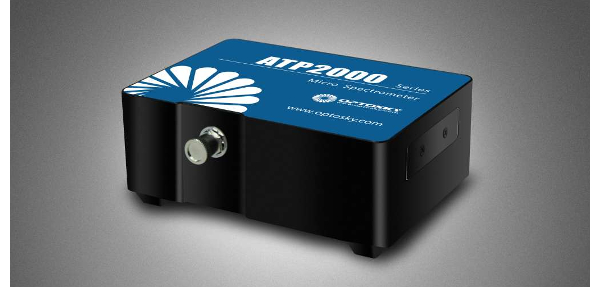
They are used as analytical tools in R&D and manufacturing laboratories as well as in the field to determine concentrations of an element in substances, identify contaminants, check for counterfeit or illegal substances or objects, analyze biological compounds, identify chemical properties of compounds, perform medical analysis, and identify structural defects among a host of others.
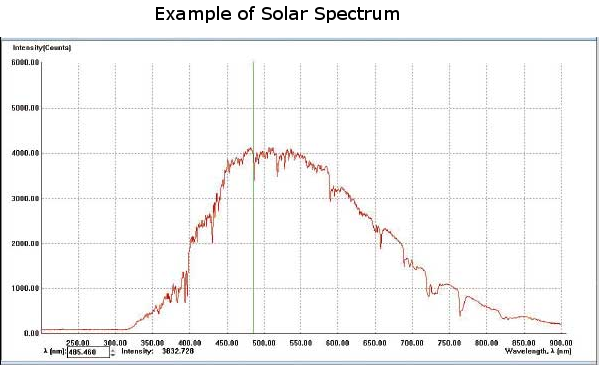
How Does a Spectrometer Work?
The basic question here is how do you separate light into it’s component wavelengths? Then how do you measure that result?
A brief discussion of the diffraction is in order here to understand how a spectrometer works. Diffraction of light is defined as the bending of light waves around an obstacle (aperture or slit), whose dimensions are comparable to the wavelengths of the incident light, and the spreading out of that light beyond the aperture. That’s only part of the story, add more apertures, thousands more. Because of the path length each wave travels to an arbitrary point in space from each aperture, and because of constructive and destructive interference at those points, the light waves are spatially separated into integer multiples of wavelengths (constructive interference) and dark spaces (destructive interference) satisfying the diffraction equation:
dsin(Θ) = mλ
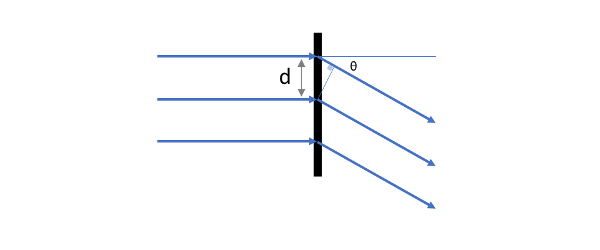
- d - is the distance between apertures
- Θ - is the angle between the light ray path to the point in space and the plane of the aperture
- m - is an integer number
- λ - is the wavelength
Replace the slits with parallel, angled grooves and you have a modern diffraction grating (an old technology, the compact disc, can be used as a diffraction grating!).
Light, then, whether directly or through a medium is directed along an optical path inside the spectrometer through an entrance aperture, on to specialized optical components to filter (in some cases) and collimate the light, then directing it onto the diffraction grating which spatially separates the light into its component wavelengths and finally directs these spectral components to a focal plane or point where a single detector or detector array is located. Once the light is imaged onto the detector array or detector it is converted to an electrical current which is then digitized usually through an on-board analog to digital converter. The digitized signal is then transmitted to a computer and processed through specialized algorithms which enables it to be plotted as a function of each individual wavelength over its spectral range.
Below is a good video for further information on how a fiber optic spectrometer works.
Main Components of a Spectrometer
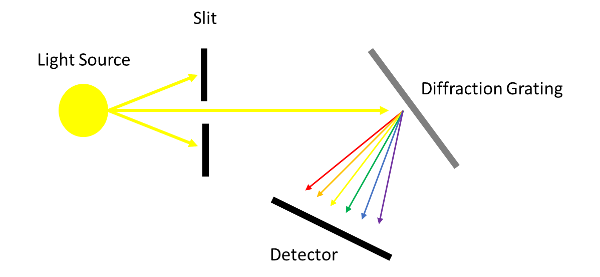
Light enters the spectrometer through a narrow vertical slit on the order of the wavelength of the light to be measured. The slit width determines how much light (intensity) enters the spectrometer and determines the resolution of the spectrometer. The narrower the slit, the higher the resolution (the differences in wavelength that the spectrometer can resolve).
The next major component is the diffraction grating. The simplest and least expensive diffraction grating is the ruled grating. This grating consists of a substrate with a large number of etched parallel angled grooves coated with a highly reflective material.
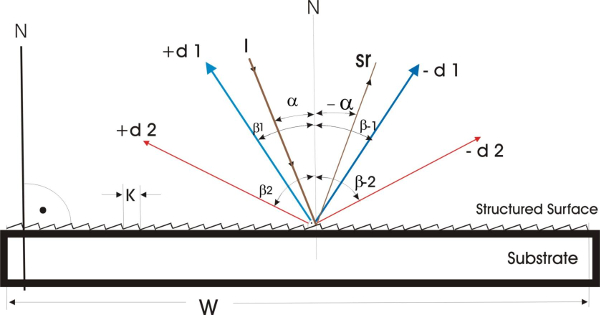
The density of the grooves determines the spectrometers wavelength coverage. Spectrometer designs use ruled gratings because of flexibility, they can operate in the 50 nm to 50 micron wavelength range (50 to 3600 grooves/mm). A holographic grating functions similarly but uses a periodic (sinusoidal) change in the index of refraction of a coated glass substrate to separate the incident light into its components. Since holographic gratings are produced optically, groove form and spacing are more consistent thus producing a more uniform spectral response and a much higher angular dispersion. These are designed to perform well over a more fixed spectral range, not in applications requiring the need to change gratings.
The University of Virginia has a more in depth presentation on diffraction gratings if you need to learn more.
The separated light is directed to a photodetector or a detector array located at the image point or plane. In compact UV to infrared spectrometers, there are two types of detectors differentiated by its material, silicon (Si) for short wavelength measurements, and InGaAs for long wavelength measurements. There are single detector spectrometers, CCD (charge-coupled device) and PDA (photo-diode array) spectrometers. With a single detector, the diffraction grating is rotated to image the incident light onto the detector element. With a CCD and photodiode detector array, the diffraction grating is fixed; each component of the diffracted light is imaged onto a respective array element, each pixel (or element) represents a portion of the spectrum. These instruments have advantages over a single detector instrument where there are no moving parts which reduce the cost, the size and the power consumption especially beneficial for field use spectrometers.
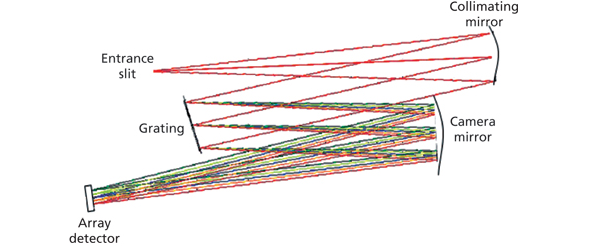
Spectrometer Optical Paths
The function of the optical components of the spectrometer is to image the entrance slit onto the detector or detector array. The spatial transmission of the light directed through the spectrometer by optical components is called the optical path. There are many path configurations in laboratory and field spectrometers depending on the location of the components, the properties of the components (size and focal), the available space (size of the instrument) each with respective bandwidth (spectral coverage), resolution and throughput characteristics. The following sections describe the Czerny-Turner optical path found in most compact and miniature spectrometers.
Czerny-Turner Optical Configuration - This article thus far has been describing a Czerny-Turner configuration of two concave mirrors, positioned for collimating the light from the entrance slit onto the diffraction grating, then focusing the reflected light onto the detector. A significant advantage of this system is that relative high performance in a low-cost compact or miniature spectrometer can be achieved by implementing low cost elements. The design is flexible and easily configurable by substituting a range of entrance slits, lenses, diffraction gratings and detectors for the many various applications it is intended for. This design delivers a flat focal plane ideal for CCD detector arrays. Two configurations are the Crossed Czerny-Turner and Unfolded Czerny-Turner. The spectrometers configured in this way deliver low to medium spectral resolution with low to medium signal to noise ratio and high throughput especially when configured with a CCD or photodiode array.
Crossed Czerny-Turner Optical Configuration - This configuration is characterized by a more compact optical path geometry; folded or crossed optical paths between the collimating mirror, diffraction grating, focusing mirror and detector. But because of its crossed configuration aberrations at the detector show up as a broadening of the image decreasing the spectral resolution. Typical resolutions are on the order of 0.5 to 10 nanometers in miniature spectrometers. To minimize these aberrations, the design of the optical path characteristics is optimized by application. This is generally done through selection of the optical systems lenses with specific size and focal lengths. It is not the intention of this article to dive into these specifics, however, in general, to reduce the system aberrations, the optical path is designed with higher F/# which is typically specified on the respective data sheets. It is presented here to alert the reader of the importance and effect on the system. There are trade-offs however with this approach in the optical power gathering capability of the system, hence an effect on throughput and signal to noise ratio. Another parameter to be aware of is numerical aperture which is the light acceptance angle of fibers and optical lenses. In the crossed configuration, there is inherent stray light from reflections in the system. A mismatch in the numerical aperture between the fiber optic input and the collection optics results in fairly high levels of stray light. This results in optical noise in the system thus decreasing the signal to noise ratio. The signal to noise ratio of these systems can be in the realm of 250:1.
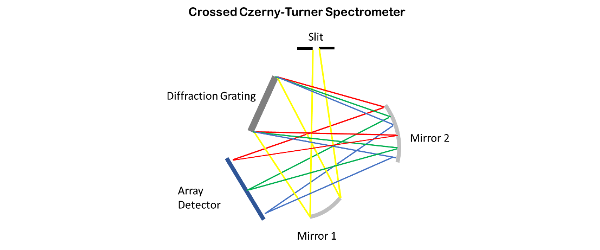
Unfolded Czerny-Turner - One way to get around the inherent stray light of the crossed Czerny-Turner configuration is by unfolding the optical path by re-positioning the collimating and focusing mirrors. This allows for beam blocks (black body or absorbers) to be inserted in the optical path to reduce the stray light. This common configuration is known as the Unfolded Czerney_Turner or “W” configuration as the path of the light would suggest. This configuration with the beam blocks reduces the optical noise increasing the signal to noise ratio especially beneficial in low-light level ultra-violet applications.
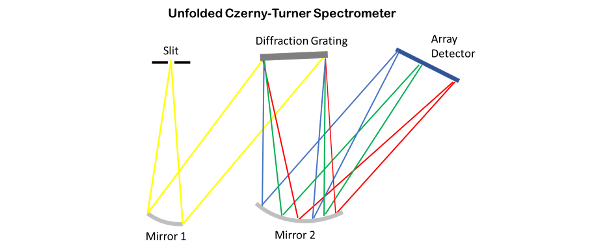
Concave holographic spectrographs - The third most common optical bench is based on the concave holographic grating, where the diffraction grating not only separates the incident light into its component wavelengths, it also focuses the separated light onto the detector array. Consequently, there are less optics in this design which helps reduce stray light, up to 10X. The only source of stray light is from the grating. Even so, because of the optically based manufacturing process of the grating, mechanical irregularities are minimal reducing the stray light. For applications such as Raman spectroscopy, where signal-to-noise is critical, the inherent low stray light of a holographic grating is an advantage. Concave aberration corrected gratings allow lower F/# optical paths meaning greater light collection capability, F/2 is common where in the ruled gratings, F/3 – F/5 is common. Holographic grating-based spectrometers are characterized by higher throughput, excellent wave front flatness, high wavelength resolution and high wavelength dispersion.
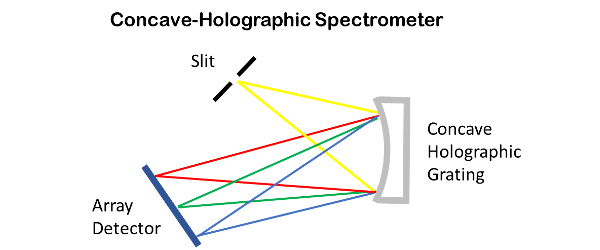
What is Fluorescence Spectroscopy?
We talked earlier about spectroscopy being a scientific measurement technique that investigates and quantifies the interaction of a light source with a sample. Fluorescence spectroscopy is an analytical method based on the fluorescence of a chemical sample, a phenomenon where a molecule absorbs light within its absorption band and then emits this light at longer wavelengths. Light of a specific wavelength band, called excitation wavelengths, is passed through a solution where it is absorbed by molecules called fluorophores, not all molecules fluoresce, hence, a unique identifier allowing the detection of specfic compounds or concentration of these compounds.
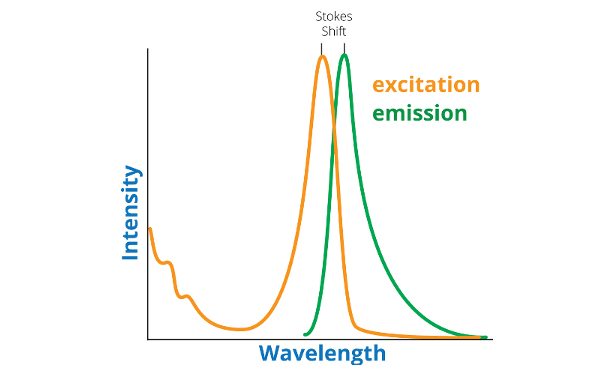
A fluorescence spectrometer, configured appropriately with filters (wavelength selecting lenses) and a sample holder is used to measure which wavelengths are absorbed by the sample and which wavelengths are emitted by the sample resulting in an excitation and emission spectrum. The spectrometer is configured with a high energy light source in the UV (ultra-violet) or visible spectrum from a laser, LED or gas lamp. The light is filtered appropriately to select the wavelength band, broken up into its constituent wavelengths and the resulting emission by the sample is measured by the detector, a photomultiplier tube which amplifies the electrons generated by a photon. Typically, the sample is scanned across the spectrum of the light source for a complete spectral analysis of the sample.
This technique is widely used for measuring compounds that can be dissolved in a solution in biochemical, medical and chemical research fields and used to identify toxins in forensic and security applications.
What is Absorption Spectroscopy?
Absorption spectroscopy differs from Fluorescence spectroscopy in that it uses the wavelength dependent absorption characteristics of molecules in a sample to identify specific elements or compounds and quantify how much is present. The absorbed light isn’t wavelength shifted and re-emitted. In absorption analysis, a comparison of the intensity of a beam of light measured before and after interaction with a sample is quantified, the photons the atoms absorb and emit are identical. There are different types of absorption spectroscopy; ultraviolet or UV, visible, infrared, and microwave. UV and visible light absorption change the molecule’s electrons energy state moving them from the ground level to a higher energy level. Infrared and microwave radiation excites vibrations of the molecules. Elements in the periodic table each have characteristic energy-level structures. The kinds of transitions the electrons can make due to its structure and therefore the energy they can absorb is unique to the element. As the electrons settle back to their initial energy or vibrational state, the resulting spectrum acts like a fingerprint uniquely identifying the element.
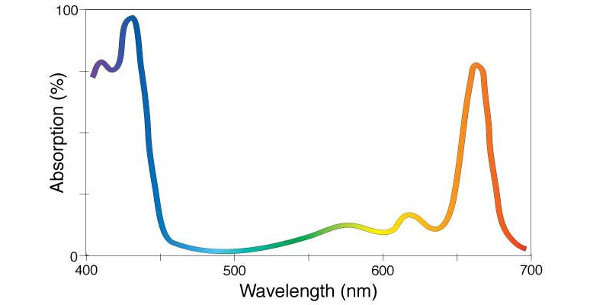
An absorption spectrometer differs from a fluorescence spectrometer. The sample is drawn into the instrument to a nebulizer, where the sample becomes fine droplets. A flame further breaks down the sample into free atoms and ions. A source light in a band that is particular to the element is directed through the sample, some of or a range of wavelengths is absorbed by the sample, the others are passed through. The light then passes through the spectrometer, is broken up into its constituent wavelengths and resulting emission by the sample is measured by the detector to produce an excitation and absorption plot.
Absorption spectroscopy is one of the most widely used in physics, chemistry and industry for medical analysis, environmental analysis, pharmaceuticals and material analysis. Infrared spectroscopy is used in gas analysis and identification of chemical structures, UV and visible spectroscopy are used for quantitative analysis of atoms, ions and chemical species in a solution.
An interesting read from NASA titled The Full Palette of Photosynthesis; about how plants absorb specific wavelengths and how plants on other planets may have a different color based upon the output spectrum of their star. This can be proven using absorption spectroscopy.
What is Raman Spectroscopy?
Raman spectroscopy is a light scattering technique. In this spectroscopy, the sample is illuminated with a monochromatic light source, usually from a laser, which interacts with the molecules of the sample scattering light. Much of this scattered radiation has a frequency which is equal to the frequency of incident radiation known as Rayleigh scattering. Only a small fraction of the scattered radiation has a frequency different from the frequency of the incident radiation (the Raman Effect) and constitutes Raman scattering. Raman scattering can be classified as two types, Stokes Raman and anti-Stokes Raman scattering. When the frequency of the scattered radiation is lower than the incident radiation Stokes lines appear, when the frequency of the scattered radiation is higher than the incident radiation, anti-stokes lines appear in the Raman Spectrum. This scattered light is used to construct a Raman spectrum, presented as an intensity vs wavelength shift and expressed as a wavenumber (inverse of wavelength). An analysis of the Raman spectrum in terms of wavenumber, peak intensity, width and shift (either direction) are tell-tales signs of foreign material, crystal structure, stress and strain at the crystal level and direction and orientation of molecules.
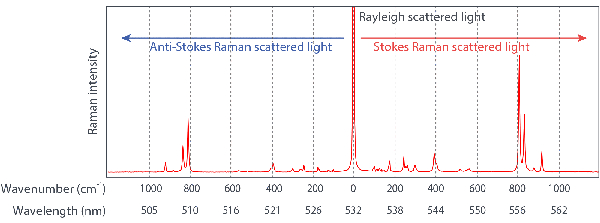
A Raman spectrometer are similar to a fluorescence spectrometer in that optical filters are incorporated to separate the relatively weak Raman scatter from the more intense Rayleigh scatter radiation. The scattered light collected from the sample is directed through the spectrometer’s the optical path, separated by wavelength and focused on a detector array. The information is processed into a spectrum which displays the intensity of light as a function of wavenumbers.
Raman spectroscopy is used where non-destructive, chemical analysis and imaging is required in both R&D and QA/QC in a variety of industries and academic fields such as semiconductors, polymers, pharmaceuticals and cosmetics, geology and minerology, life sciences (DNA/RNA analysis for example), semiconductor manufacturing, safety and security among others.
An interesting example of Raman Spectroscopy is provided by Jet Propulsion Laboratory and California Institute of Technology where they built a a mini time-resolved Raman spectrometer for planetary science based on an avalanche diode detector array.